Green Batteries: The Sustainable Future of Energy Storage
- Nexxant
- May 22
- 18 min read
Introduction
As the world accelerates its transition to renewable energy, sustainable energy storage has become one of the greatest challenges of our time. It’s impossible to talk about solar, wind, or hydro power at scale without addressing how that energy will be stored safely, affordably, and in an environmentally responsible way.
For years, lithium-ion batteries have dominated the energy storage landscape — but the environmental and social costs of their production, combined with the scarcity of key raw materials like cobalt and nickel, have raised concerns across the industry. In response, a new paradigm is emerging: green batteries.
More than just a technological replacement, these batteries represent a structural shift. They are designed to minimize environmental impact from material extraction to end-of-life disposal, adopting safer, recyclable, and more abundant alternatives. Technologies such as sodium-ion, solid-state, organic, air-based, and saltwater batteries are at the forefront of this revolution — each offering unique benefits, challenges, and promising use cases.
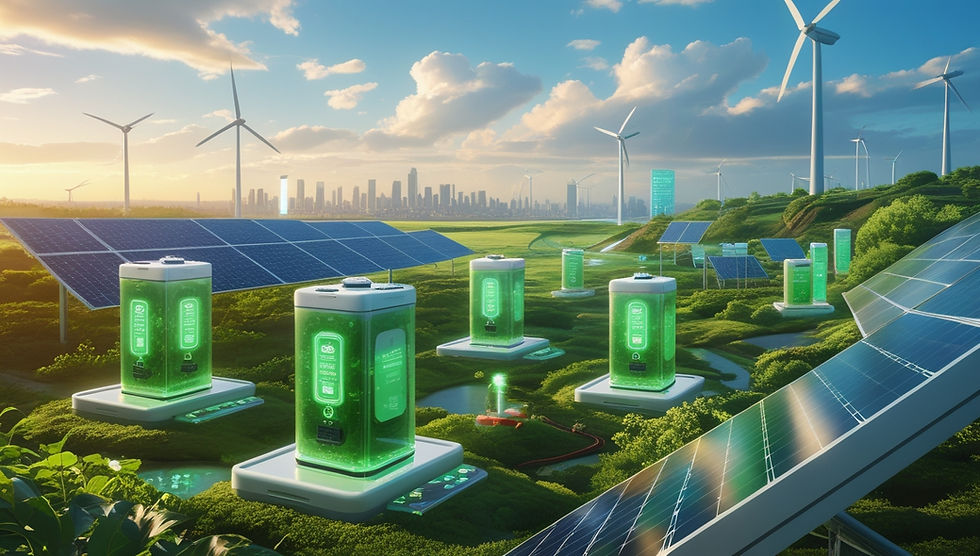
In this article, you'll discover what green batteries are, how they work, their key advantages and disadvantages, and the current stage of their technological development. We’ll also explore the opportunities and challenges for large-scale adoption of these emerging battery technologies, taking into account real-world applications, market projections through 2030, and the social and environmental impacts of this new energy frontier.
Whether you're a researcher, industry professional, investor, or simply someone keeping an eye on the future of energy, this article offers a comprehensive — and realistic — overview of lithium alternatives and their role in shaping the next generation of sustainable energy storage.
2. What Are Green Batteries?
As the world shifts toward a cleaner energy model, the demand is growing for solutions that not only store energy efficiently but also respect the planet’s ecological boundaries.
But what exactly defines a battery as “green”?
2.1 Definition and Core Principles
Green batteries are energy storage systems designed with sustainability as a core principle throughout their entire lifecycle — from sourcing to operation to disposal. They aim to mitigate the environmental damage associated with conventional batteries like lithium-ion, which, while efficient, rely on intensive mining, generate toxic waste, and pose risks of fire and explosion.
Unlike mainstream battery technologies, green batteries don’t just represent a technological evolution — they propose a paradigm shift, incorporating principles of circular economy, biomimicry, low ecological impact, and improved ecosystem compatibility.
2.2 What Makes a Battery “Green”?
To be considered part of the green battery family, a technology must meet key sustainability criteria, including:
Low environmental impact during production: The use of less toxic, biodegradable, or widely available materials — such as sodium, zinc, carbon, or organic compounds — reduces environmental degradation and the risks tied to mineral extraction.
Abundant and recyclable materials: Technologies like sodium-ion batteries use elements that are plentiful in the Earth’s crust, reducing dependence on scarce minerals such as cobalt or even lithium.
Safety and low toxicity: The absence of heavy metals or flammable electrolytes makes these batteries safer for both residential and industrial applications. Some solid-state and saltwater-based batteries stand out for their safety profiles.
High energy efficiency with a lower carbon footprint: While not all green battery technologies match lithium-ion in raw performance, many offer longer lifespans, lower degradation rates, and easier recyclability — contributing to sustainable energy storage over time.
2.3 Comparison with Conventional Batteries
Conventional batteries — like lead-acid and lithium-ion — have dominated the market for decades. Despite their efficiency, they come with significant environmental costs. Lithium mining, for instance, consumes vast amounts of water and energy, exacerbating water scarcity in regions like the Lithium Triangle (Argentina, Bolivia, and Chile). Meanwhile, improper disposal of lead-based batteries remains a public health issue in many developing countries.
In contrast, emerging battery technologies — especially those based on organic compounds, sodium, or aqueous electrolytes — offer low-carbon alternatives to lithium with greater recyclability and lower ecological impact.
These sustainable battery technologies, intended for electric vehicles, grid-scale storage, and stationary applications, are still under development but are already gaining traction. Leading institutions like the Fraunhofer Institute (Germany), the Argonne National Laboratory (USA), and startups such as Natron Energy and Form Energy are pioneering innovations in clean energy storage with reduced environmental impact.
3.0 Technological Landscape: The Main Alternatives to Lithium
In this chapter, we explore the leading emerging battery technologies that form the ecosystem of so-called green batteries — alternatives that aim to reduce environmental impact without compromising energy efficiency.
Each approach offers distinct advantages, technical challenges, and varying degrees of technological maturity. Let’s take a closer look at how sodium-ion, solid-state, organic, air-based, saltwater, and other experimental battery solutions work.
Technology | Main Advantages | Current Maturity Stage |
Sodium-ion | Low cost, abundant materials, good cold-weather performance | Pilot projects and early factories |
Solid-State | High energy density, no explosion risk | Pre-commercial (2027–2030 expected) |
Organic | Biodegradable, no heavy metals | Lab-scale and niche applications |
Air (Li-Air, Zn-Air) | Extremely high density, uses ambient oxygen | Prototypes (Li), niche use (Zn) |
Saltwater | Non-toxic, highly safe, ideal for off-grid storage | Field testing and pilot deployment |
3.1 Sodium-Ion Batteries: The Most Accessible Element on the Periodic Table
Among all current lithium alternatives under development, sodium-ion batteries stand out for a simple reason: sodium is one of the most abundant elements on Earth. Easily found in salt deposits and seawater, it offers a potentially lower-cost and more eco-friendly solution compared to conventional lithium-ion batteries.
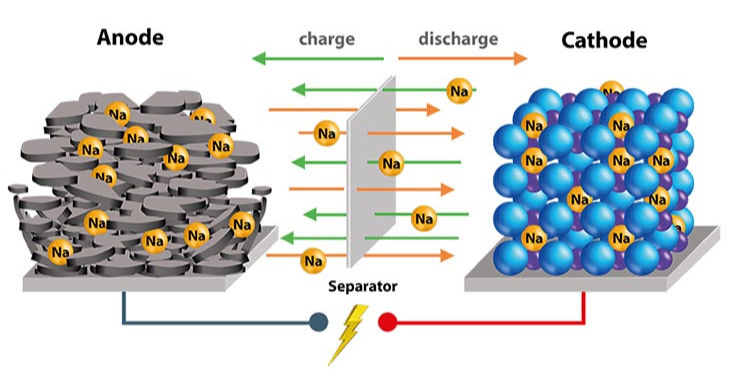
How Sodium-Ion Batteries Work
Sodium-ion batteries operate similarly to lithium-ion batteries, with ions moving between electrodes during charge and discharge cycles. The key difference lies in the active material: while lithium is a lightweight and highly reactive metal, sodium is larger and heavier — which directly impacts the energy density of the cell.
A typical structure includes a carbon-based anode (often hard carbon) and a cathode made from sodium oxides or phosphates. The electrolyte is usually an organic solvent with dissolved sodium salts, although research into aqueous electrolytes is advancing for low-voltage applications.
Advantages of Sodium-Ion Batteries
Low cost and high availability: Sodium is abundant and inexpensive, potentially reducing production costs compared to lithium.
Sustainability and reduced environmental impact: These batteries avoid intensive mining and rare metals, contributing to a more sustainable energy storage model.
Good performance in low temperatures: Makes them useful in colder climates where lithium batteries underperform.
Compatibility with existing manufacturing infrastructure: Many production processes are similar to lithium-ion, enabling a smoother transition.
Disadvantages and Limitations
Lower energy density: Sodium-ion batteries currently lag behind lithium-ion in terms of energy stored per volume or weight.
Limited cycling in certain compositions: Some cathode materials degrade faster, reducing battery lifespan.
Miniaturization challenges: Not yet viable for compact applications like smartphones or drones.
Recent Developments and Leading Innovators
In 2021, China’s CATL (Contemporary Amperex Technology) announced its first commercial-scale sodium-ion battery, aiming to integrate it into electric vehicles and hybrid systems alongside lithium batteries. In the U.S., Natron Energy is developing sodium-ferrocyanide batteries for industrial and stationary use, focusing on durability and safety.
Institutions like the Argonne National Laboratory (USA) and the Dalian Institute of Chemical Physics (China) are leading research into advanced cathode materials and electrolytes to boost the performance of next-generation eco-friendly sodium-based batteries.
Maturity Level
Sodium-ion batteries are currently transitioning from lab-scale research to pilot production. While they have yet to achieve mass-market penetration, they’re emerging as one of the most promising next-generation battery technologies for medium-scale applications such as energy backup, stationary storage, and electric public transit.
Though not yet ideal for most electric vehicles, steady progress in electrochemical composition and system engineering suggests that their role will expand significantly in the coming decades — particularly in markets seeking scalable and affordable energy solutions.
3.2 Solid-State Batteries: Safety and Efficiency Beyond Conventional Lithium
Widely regarded as one of the most promising emerging battery technologies, solid-state batteries represent a major technical leap over traditional liquid-electrolyte models. Their primary appeal lies in the combination of enhanced safety, higher energy density, and compatibility with advanced conductive materials — including metallic lithium itself.
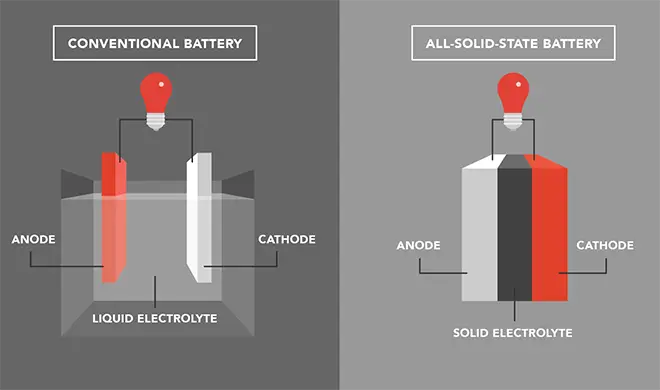
How Solid-State Batteries Work
The key difference from conventional lithium-ion batteries is the replacement of the flammable liquid electrolyte with a solid-state electrolyte. This solid layer can be made of a conductive polymer, ionic ceramic, or glassy compound, and performs the same function of transporting ions between electrodes during charge and discharge cycles.
This architecture not only eliminates the risk of leakage and combustion but also allows for the use of ultra-thin metal anodes, significantly increasing energy density — a critical feature for applications in electric vehicles, light aviation, and compact electronics.
Advantages of Solid-State Batteries
Enhanced thermal and structural safety: The absence of flammable liquids drastically reduces the risk of explosion and overheating.
Superior energy density: Capable of storing up to twice the energy of lithium-ion batteries at a smaller volume and weight.
Longer lifespan: Reduced chemical degradation between components enables more stable and durable cycling.
Compatibility with new materials: Supports the use of metallic lithium and alternative compounds, potentially eliminating cobalt and nickel usage.
Disadvantages and Limitations
Manufacturing complexity at scale: Producing uniform solid electrolytes and integrating them with other components remains technically challenging.
High production cost: Materials and processing methods are still expensive, limiting mass adoption.
Moisture and temperature sensitivity: Some solid ceramics are unstable outside of laboratory-controlled environments.
Recent Developments and Key Industry Players
Major companies such as Toyota and QuantumScape (backed by Volkswagen and Bill Gates) have made substantial progress toward the commercial viability of solid-state batteries. QuantumScape, for example, has announced prototypes capable of reaching 80% charge in under 15 minutes, while maintaining cell integrity for over 800 cycles — a key milestone for the automotive industry.
In Europe, Solid Power, supported by BMW and Ford, is heavily investing in scaling up production, focusing on electric vehicle batteries with longer range and improved thermal stability.
Leading research institutions such as MIT, Fraunhofer Institute, and the Samsung Advanced Institute of Technology are spearheading studies on new ceramic and hybrid electrolytes, as well as automated manufacturing techniques that could reduce costs and make the technology more accessible in the coming years.
Maturity Level
Despite its vast potential, solid-state battery technology still faces technical and economic barriers to mass production. According to industry experts, a realistic timeline for commercial-scale deployment is between 2027 and 2030, initially targeting high-value niches such as premium electric vehicles, industrial drones, and military applications.
From an environmental standpoint, while some models still use lithium, the removal of toxic electrolytes and the potential to eliminate cobalt make solid-state batteries strong candidates for integration into the green battery ecosystem.
3.3 Organic Batteries: Sustainable Energy from Living Molecules
In a world increasingly driven by environmental goals, organic batteries emerge as a radically different promise. Instead of relying on heavy metals or toxic electrolytes, these systems use abundant organic compounds — many inspired by biomolecules found in nature — to store and transfer electrical charge. It’s the fusion of green chemistry with electrochemical innovation.
How Organic Batteries Work
Unlike traditional batteries that depend on metallic ions like lithium or sodium, organic batteries utilize electron-rich molecules such as quinones, phenols, or redox polymers, which undergo reversible reactions during charge and discharge cycles. These reactions occur on lightweight, flexible electrodes, often paired with aqueous electrolytes or non-toxic solvents.
One particularly promising branch is organic redox flow batteries, where the active organic material is dissolved in a liquid electrolyte and stored in external tanks. This enables scalable flexibility — ideal for stationary energy storage and integration with other renewable energy systems.
Advantages of Organic Batteries
Abundant and renewable raw materials: Built from elements like carbon, hydrogen, and oxygen, often sourced from plant-based or synthetic organic compounds.
Extremely low environmental impact: By definition, they are eco-friendly batteries, and can be manufactured using recyclable and biodegradable components.
Chemically customizable: The molecular structure of organic compounds can be tailored to optimize voltage, capacity, or stability.
Compatible with aqueous electrolytes: Reduces fire hazards and simplifies disposal and recycling processes.
Disadvantages and Challenges
Limited stability: Many organic molecules degrade over time, especially when exposed to oxygen or light.
Lower energy density: Compared to lithium-ion or solid-state batteries, they store less energy per volume.
Industrial scale-up hurdles: Most projects are still in prototype or lab development stages.
Key Research and Initiatives
Leading the development of organic battery technologies is the Harvard John A. Paulson School of Engineering and Applied Sciences, which has advanced redox flow batteries using non-toxic organic compounds with durability exceeding 1,000 cycles. Meanwhile, Swiss startup Battrion is investing in organic polymer-based batteries for lightweight, flexible use in electronics and wearable sensors.
Other innovative efforts include the use of lignin derivatives — a byproduct of the paper industry — as a base for conductive and sustainable electrodes, turning industrial waste into energy assets.
Researchers at the Fraunhofer Institute (Germany) and the University of Tokyo are also working on integrating redox polymers with 3D carbon frameworks to enhance charge density and extend cell life.
Maturity Level
Despite their environmental appeal, organic batteries remain at a pre-commercial stage. Their most immediate applications are in stationary energy storage and low-power electronics, but continued progress in molecular stability and electrode engineering may enable large-scale adoption in the medium term.
These solutions are gaining attention in the green battery ecosystem by offering a viable path toward a supply chain free from toxic or rare metals — making them natural allies of solar and wind energy systems.
3.4 Air Batteries: High-Density Energy from the Air We Breathe
Among all emerging battery technologies, air batteries — particularly lithium-air and zinc-air — are frequently cited as candidates for a revolution in the energy sector. Their unique advantage lies in the ability to use ambient oxygen as a reactant, potentially reducing battery weight and significantly increasing energy density. However, their promise is still constrained by serious technical challenges.
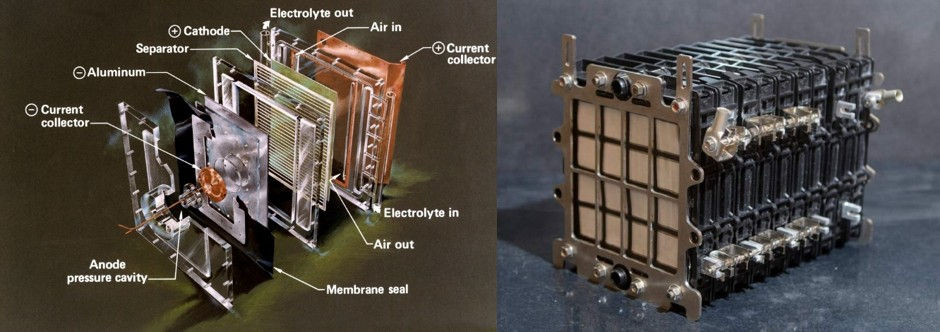
How Air Batteries Work
The core principle behind air batteries is a redox reaction between a metal (such as lithium, aluminum, or zinc) and oxygen from the surrounding air. In lithium-air batteries, the anode is made of metallic lithium, and the cathode is porous to allow atmospheric oxygen to enter. During discharge, lithium reacts with oxygen to form peroxides or oxides, releasing electrical energy.
Zinc-air batteries, on the other hand, use metallic zinc as the anode and a catalytic air electrode that absorbs oxygen to generate current. These are already used in applications like hearing aids and sensors, but the goal is to scale them up for electric vehicles and long-range drones.
Advantages of Air Batteries
Extremely high energy density: In theory, lithium-air batteries can reach energy densities close to that of gasoline — around 1,000 Wh/kg, far beyond today’s lithium-ion batteries (~250 Wh/kg).
Lightweight design: Since oxygen is sourced from the environment, part of the battery volume is eliminated, reducing weight and complexity.
Simplified component structure: Fewer internal materials may streamline long-term manufacturing.
Use of abundant and less toxic materials (zinc): Zinc is more common and less hazardous than lithium, positioning zinc-air batteries as potential eco-friendly energy storage options.
Disadvantages and Limitations
High chemical instability (lithium-air): Reactions with oxygen can create highly reactive compounds that rapidly degrade electrodes.
Short lifespan: Many prototypes fail after just a few dozen cycles.
Dependence on costly catalysts: Rechargeable zinc-air batteries often require noble metals like platinum to facilitate reactions.
Sensitivity to humidity and CO₂: Exposure to atmospheric contaminants can compromise cell performance.
Recent Advances and Key Researchers
In the lithium-air field, the Argonne National Laboratory (USA) is leading efforts to stabilize electrolytes and electrodes, focusing on moisture- and CO₂-free environments. In 2023, researchers at the University of Illinois Chicago reported promising progress using graphene-based catalysts to improve lithium-oxygen reaction reversibility.
For zinc-air systems, Canadian startup Zinc8 Energy Solutions has developed a scalable energy storage platform for power grids, using regenerable zinc and separating reaction zones into modular systems — ideal for renewable energy storage in remote or under-resourced areas.
Additionally, NantEnergy has been testing zinc-air batteries in telecommunications and public lighting applications, particularly in developing countries, as part of low-cost, sustainable energy storage projects.
Maturity Level
Air batteries, especially lithium-air variants, are still far from commercial viability. Despite promising lab results, chemical instability and limited durability restrict their use to prototypes and tightly controlled experiments. Zinc-based versions — simpler and more affordable — already see commercial use in niche applications like medical devices and small-scale stationary systems.
Within the green battery ecosystem, this class represents more of a future innovation frontier than a mass-market-ready solution. Still, if key obstacles are overcome, air batteries could completely redefine the concept of portable, clean energy, particularly in scenarios where weight and energy density are critical.
3.5 Saltwater Batteries: Safety and Sustainability with Natural Electrolytes
In a world where sustainability and safety are increasingly non-negotiable, saltwater batteries — also known as aqueous electrolyte batteries — are gaining attention as an environmentally responsible alternative. This technology embraces simplicity: replacing flammable organic electrolytes with water-based solutions containing dissolved salts, typically sodium chloride (NaCl) — table salt.
While still niche in the market, these batteries represent a significant step forward in the development of green battery technologies, combining low cost, high safety, and minimal environmental impact in an architecture well-suited for sustainable energy storage.
How Aqueous Electrolyte Batteries Work
The operating principle is similar to other rechargeable batteries: ions move between the anode and cathode through the electrolyte, generating electric current. The key difference is the composition — instead of toxic organic solvents, a water-based solution with dissolved salts like NaCl or ZnSO₄ is used. The result is a cell with significantly lower thermal and environmental risks.
Common configurations include electrodes made of carbon, metal oxides, or hybrid compounds containing zinc, manganese, or iron, offering a broad range of applications.
Advantages of Saltwater Batteries
Exceptional safety: Based on water, they are non-flammable, eliminating risks of explosion, toxic leakage, or overheating.
Abundant, non-toxic materials: Using table salt and common metals drastically reduces costs and environmental impact, aligning with the ethos of eco-friendly batteries.
Easy to dispose and recycle: The absence of heavy metals and dangerous solvents simplifies end-of-life processing.
Ideal for stationary storage: Applications such as microgrids, solar farms, and off-grid systems benefit from their durability and safety.
Disadvantages and Challenges
Low energy density: These batteries store less energy per unit of mass or volume compared to lithium- or sodium-based technologies.
Limited operating voltage: Water has a narrow electrochemical window (~1.23V), restricting performance potential.
Durability concerns: Electrode corrosion and side reactions with water can still shorten battery lifespan in some designs.
Key Innovations and Research Highlights
In 2021, the Chinese Academy of Sciences published a study on zinc-ion aqueous batteries using polymer gel anodes and manganese oxide cathodes, achieving over 500 cycles with high stability and no thermal risk — a milestone for residential applications.
Swiss startup Green Energy Storage is testing saltwater battery prototypes for large-scale storage in industrial and agricultural settings. In Brazil, researchers at UFMG and USP are developing low-cost prototypes using saltwater and recycled materials like graphite and iron.
Collaborative projects between Stanford University and ESS Inc. (known for its iron-flow batteries) are exploring alkaline aqueous electrolytes to improve durability and make these solutions viable for smart grid integration.
Maturity Level
Saltwater batteries already have working prototypes with practical applications in small-scale renewable energy storage systems. They are not yet suitable for electric mobility or portable electronics, where high energy density is required, but they show strong promise for stationary use cases, including home backup systems, solar energy storage, and off-grid rural networks.
Within the next generation of sustainable batteries, aqueous systems stand out for their extreme safety and environmental appeal, positioning them as strategic tools for decentralizing and democratizing access to clean energy — especially in regions where cost and risk remain major barriers.
3.6 Other Experimental Technologies: The Living Lab of Future Green Batteries
While sodium, solid-state, and saltwater batteries are gaining traction in the energy sector, numerous other approaches remain at the laboratory stage, aiming to disrupt paradigms in the search for more sustainable, safer, and more efficient energy storage. Though not yet commercially viable, these experimental technologies offer promising pathways within the broader field of green battery research.
Carbon-Based Batteries: Lightweight, Conductive, and Potentially Renewable
Researchers at Chalmers University of Technology in Sweden are developing structural carbon batteries — materials that can simultaneously store energy and serve as structural components in electronic devices. These carbon batteries explore graphene, carbon nanofibers, and conductive polymers to create ultra-light and flexible electrodes.
The key innovation lies in their sustainability and multifunctionality: carbon can be sourced from renewable materials such as plant waste or even captured directly from the atmosphere (via CO₂ capture), contributing to a clean and intelligent energy cycle. Despite the potential, the main limitation remains low storage capacity compared to conventional technologies.
Nanocellulose-Based Batteries: Biomaterials at the Core of Innovation
At Uppsala University, also in Sweden, researchers are pioneering batteries made from nanocellulose — a wood-derived material that is highly porous and capable of forming lightweight, biodegradable electrodes. These batteries show promise for wearable devices, disposable sensors, and medical electronics.
In addition to their ecological appeal, nanocellulose offers tunable conductivity and can be combined with metal salts to form hybrid electrode structures. However, current challenges include long-term stability and charging efficiency, limiting these batteries to prototypes in controlled environments.
Hybrid and Bioinspired Batteries
Another exciting frontier is the development of hybrid batteries that combine elements from multiple architectures — such as redox systems with solid electrodes or cells inspired by natural processes, like photosynthesis or cellular respiration. At the Karlsruhe Institute of Technology (KIT) in Germany, scientists are exploring bioinspired batteries that use hemoglobin-like molecules as charge carriers.
Other studies are investigating microbial batteries, where living microorganisms participate in electrochemical reactions, generating electricity in environments rich in organic matter — an intriguing solution for remote areas with high biomass waste.
Maturity Level
These experimental battery technologies are not yet close to commercialization. However, they play a crucial role in scientific advancement, offering alternatives that are more ecologically aligned and inspired by natural mechanisms of energy generation and storage.
Even in their embryonic stages, these innovations help expand the definition of green batteries, guided by principles of circularity, biomimicry, and responsible resource use.
4. Market and Challenges
The advancement of green battery technologies depends not only on technical innovation but also on their ability to meet real-world energy and mobility demands in a sustainable way. As governments and companies commit to decarbonization goals, the interest in energy storage solutions that offer both performance and low environmental impact is accelerating.
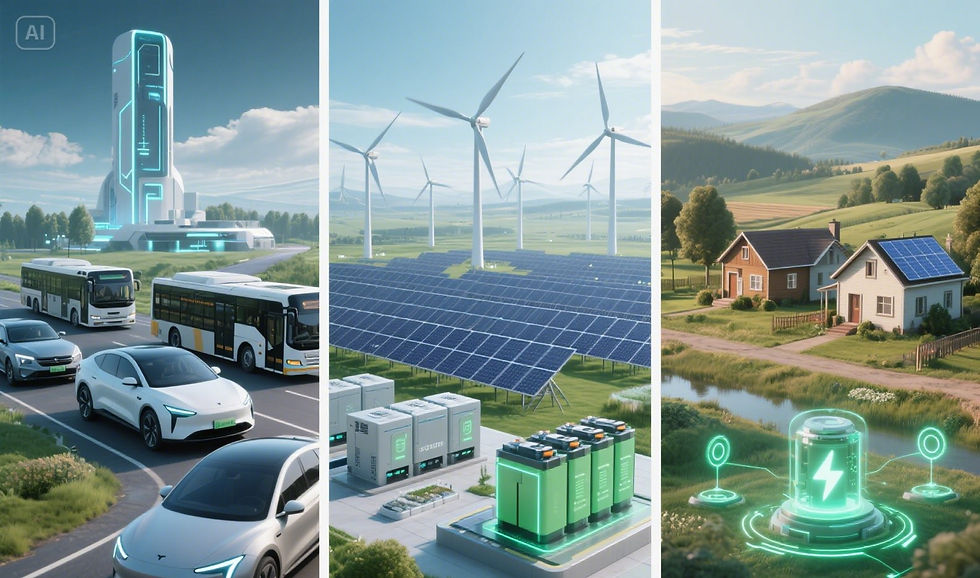
Strategic Sectors
The most promising applications for emerging battery technologies currently focus on three key areas:
Electric Mobility: Electric cars, buses, and trucks require batteries with high energy density, low weight, and enhanced safety. Solid-state batteries, and eventually air-based batteries, are strong candidates to meet this demand — especially for premium vehicles and heavy-duty transport.
Stationary Storage: To support the integration of large-scale solar and wind energy, reliable and long-lasting storage systems are essential. Sodium-ion, saltwater, and organic batteries are being tested in smart grids, solar farms, microgrids, and residential applications.
Rural Electrification and Energy Access: In remote or low-income regions with weak infrastructure, cost and safety are critical. Aqueous electrolyte batteries and hybrid green systems stand out as affordable options to democratize access to clean energy.
Startups and Key Industry Players
The green battery market has attracted a diverse ecosystem of innovators. Notable players include:
CATL and BYD (China) – commercializing sodium-ion batteries for electric vehicles and backup systems.
QuantumScape (USA) – a leader in solid-state battery development, focused on high-performance EVs.
Form Energy (USA) – specializing in grid-scale renewable energy storage using iron-air batteries.
Zinc8 (Canada) – developing modular air battery systems for telecom and community-scale energy.
Green Energy Storage (Europe) – testing organic compound batteries for industrial applications.
Market Outlook Through 2030
According to BloombergNEF, the global energy storage market is expected to grow 15-fold by 2030, reaching a cumulative capacity of over 400 GWh. The report forecasts that lithium alternatives — especially sodium, iron, and aqueous-based solutions — could represent up to 25% of stationary installations in emerging economies and logistically constrained regions.
In parallel, the European Battery Alliance and U.S. Department of Energy (DOE) are heavily investing in supply chain diversification and support for sustainable battery production for EVs and power grids.
4.2 Technological and Adoption Barriers
Despite increasing interest and research, the mass adoption of green batteries faces significant challenges. Overcoming these will be key for emerging technologies to transition from lab prototypes to widely adopted energy solutions.
Energy Density vs. Safety
There’s a persistent trade-off between safety and performance. Technologies like sodium-ion or saltwater batteries are safer but store less energy per kilogram than lithium. Conversely, air and solid-state batteries promise higher energy density but still lack the stability and reliability required for everyday use.
Cost vs. Scalability
Many alternative materials are inexpensive, but industrial scale has yet to be achieved. The lack of standardization and optimized production lines makes emerging technologies costly, even those based on abundant resources. Without government incentives or economies of scale, alternative batteries remain less competitive.
Infrastructure and Recycling
Current infrastructure is tailored to lithium-ion chemistry. Adopting new battery types will require significant adaptations in manufacturing processes, logistics, and especially in safe recycling — still underdeveloped for organic, saline, or bioinspired materials.
Regulatory and Financial Barriers
The absence of clear regulatory guidelines and policy incentives makes it difficult for many startups to raise capital or validate their products. Without standardized international certifications for green batteries, access to strict markets — such as the European Union — remains limited.
Moreover, mining conglomerates and lithium industry giants continue to influence R&D investment and infrastructure priorities, which may delay or block the mainstream adoption of lithium alternatives.
5. The Future of Sustainable Energy Storage
As the energy sector continues to evolve, green batteries are no longer just alternatives — they are emerging as central components of modern energy storage solutions. But true progress won’t come solely from incremental improvements to existing technologies. It will require deeper transformations in how we develop materials, design hybrid systems, and integrate storage with intelligent digital networks.
Research in Bioelectrolytes and Functional Materials
One of the most promising areas involves the development of bioelectrolytes — conductive solutions derived from biomaterials like amino acids, lignin, and plant-based polymers. Researchers at the University of Tokyo and MIT are working on compounds based on glycine and gelatin with stable electrochemical properties, biodegradability, and non-toxicity — a disruptive alternative to traditional electrolytes.
These materials can be combined with carbon-based electrodes or organic batteries, resulting in devices fully aligned with circular economy principles. Moreover, bioelectrolytes open the door to applications in wearables, medical devices, and smart sensors, where safety and low environmental impact are critical.
Hybrid Architectures and Multifunctional Cells
Another growing trend is the fusion of different battery technologies into hybrid systems that leverage the strengths of each. For example, some projects combine sodium-ion with air batteries for stationary applications, taking advantage of sodium’s stability and the high energy density offered by oxygen-based reactions.
Companies like Form Energy are developing battery cells that merge iron-air reactions with organic redox elements, creating customized energy solutions for everything from data centers to off-grid communities. These next-generation battery systems not only store energy but also respond dynamically to consumption patterns, acting as active agents in smart grids.
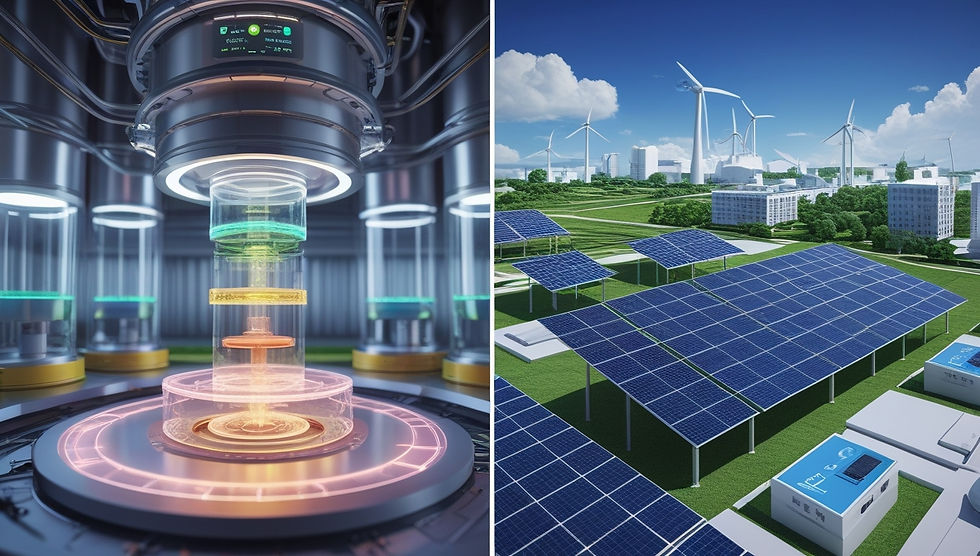
Convergence with Renewable Grids and Decentralized Energy
Energy storage can no longer be viewed as an isolated function. In a landscape dominated by intermittent renewable sources (like solar and wind), the future of energy will be distributed, automated, and intelligent. This requires batteries capable of integrating with energy management systems, weather forecasting, demand response, and automated energy trading.
The combination of green batteries with smart grids will allow homes, businesses, and cities not only to consume clean energy, but also to store and share surplus power. Initiatives like the European INTERSTORE project and the Energy Web Foundation consortium are already testing platforms that connect renewable energy storage with blockchain and IoT to enable peer-to-peer energy transactions.
The Vision of a Circular Energy Chain
At the core of this transformation is the concept of a circular energy chain, where the entire lifecycle of a battery — from raw material extraction to manufacturing, usage, and recycling — is designed to reduce impact, prevent waste, and regenerate resources.
Technologies like saltwater batteries, nanocellulose-based systems, and structural carbon batteries are moving in this direction by prioritizing local, recyclable, and renewable materials.
Conclusion
Green batteries represent more than a technological shift — they symbolize a paradigm change. In a world striving to reduce emissions, democratize energy access, and cope with the environmental limits of resource extraction, alternatives to lithium are becoming essential to reshape the global energy future.
Throughout this article, we explored how sodium-ion, solid-state, organic, air-based, and saltwater batteries stand out for their environmental promise, technical potential, and strategic applications. While many of these emerging battery technologies are still maturing, increasing investment in research, growing market diversification, and the pressure for cleaner solutions all point to a horizon where sustainable energy storage is just as vital as energy generation itself.
Now more than ever, innovation and sustainability must go hand in hand. This means supporting R&D initiatives, investing in environmentally responsible companies, and demanding public policies that accelerate the energy transition.
Enjoyed this article? Share it on social media and continue to follow us to stay tuned on the latest in AI, breakthroughs and emerging technologies.
Thanks for your time!😉
Comments