Top 5 Future Processor Technologies: From Silicon to Bioprocessors
- Nexxant
- 1 day ago
- 9 min read
Introduction
For over five decades, silicon has fueled the progress of microelectronics and served as the foundation of modern computing. However, the physical limits of traditional transistors are beginning to constrain the pace of innovation. As chip miniaturization advances beyond the nanometer scale, new technical challenges arise such as overheating, quantum noise, and rising energy consumption, which are calling Moore’s Law into question.
In response, researchers and tech companies around the world are exploring emerging processor technologies that could push the boundaries of advanced computing. The goal is not just faster computation, but the development of new computational paradigms focused on energy efficiency, architectural flexibility, and integration with intelligent systems.
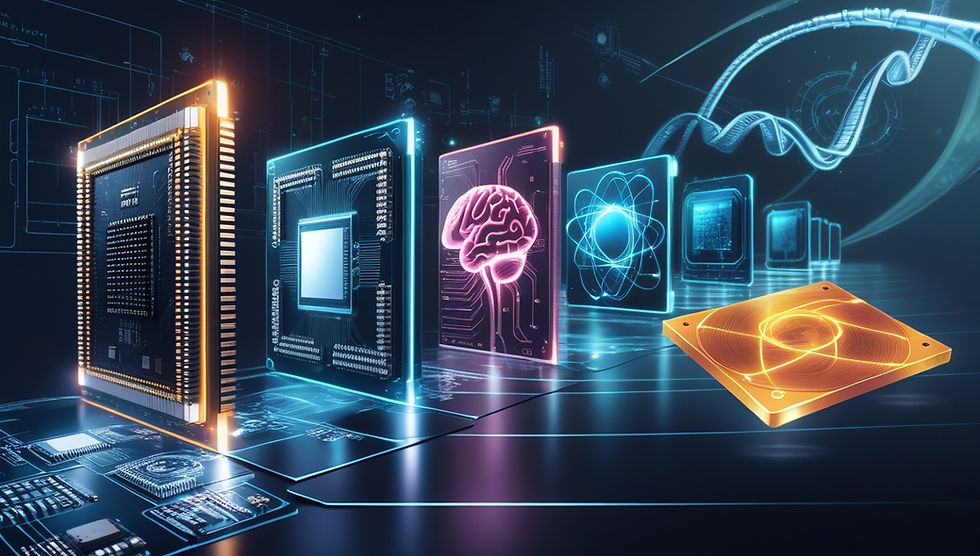
This article explores five of the most promising directions that define the horizon of future processor technologies—from photonic chips and neuromorphic architectures to bioprocessors that leverage organic molecules to process and store information. Each of these approaches addresses specific limitations of silicon and opens new paths for a computing future that is more diverse, hybrid, and adapted to the challenges of the 21st century.
1. The End of the Silicon Era?
For over five decades, silicon has reigned supreme at the heart of the digital revolution. Since the formulation of Moore’s Law in 1965—which predicted the doubling of transistor counts on a chip every two years—silicon-based chips have fueled exponential advances in advanced computing, reducing costs, sizes, and power consumption while increasing processing power.
The dominant paradigm, built around CMOS (Complementary Metal-Oxide-Semiconductor) architecture, enabled the rise of AI processors, smartphones, supercomputers, and cloud servers. However, this trajectory now faces mounting physical and economic barriers. Transistor miniaturization has reached a staggering 3 nanometers in technologies developed by TSMC and Samsung, but such progress has brought new challenges: excessive heat, quantum noise, and rising energy consumption are diminishing the marginal gains with each new chip generation.
Moreover, manufacturing costs have skyrocketed. Building a single advanced chip fabrication facility now exceeds $20 billion, requiring public-private partnerships like the U.S. CHIPS Act and strategic investments by the European Union.
Crucially, this is no longer just a technological trend—it is an urgent necessity. The growing demand for real-time computing, machine learning, and complex scientific simulations is pushing the boundaries that silicon alone can no longer overcome.
As a result, new initiatives are emerging to bypass the limits of traditional electronics, paving the way for alternative paradigms such as photonic processors, neuromorphic chips, quantum processors, and bioprocessors. These are the foundational elements of what we now call the processors of the future.
This shift isn’t simply about replacing one material with another; it involves redesigning processor architectures themselves, with deep implications for the future of computing.
The silicon era is far from over, but its undisputed dominance is being steadily challenged. What lies ahead is not a sudden rupture, but a hybrid, gradual, and profoundly disruptive transformation and it is already in motion.
2. Top 5 Future Processor Technologies
As the physical limits of silicon become increasingly evident, the need to explore emerging processor technologies is more urgent than ever to sustain the evolution of advanced computing. In this context, five promising paths are gaining traction and have the potential to reshape the sector: from photonic processors to bioprocessors, passing through neuromorphic, quantum, and 2D material-based architectures. Each of these next-generation chips offers a distinct approach to overcoming the limits of traditional silicon, with varying levels of maturity and application scope.
2.1 Photonic Processors: Computing at the Speed of Light
Photonic computing represents a radical shift in how processors operate: replacing the flow of electrons with photons (particles of light) to transmit and process data. This enables operations at speeds close to the physical limit of light, while dramatically reducing heat dissipation and energy consumption which are two of the biggest bottlenecks in silicon-based chips.
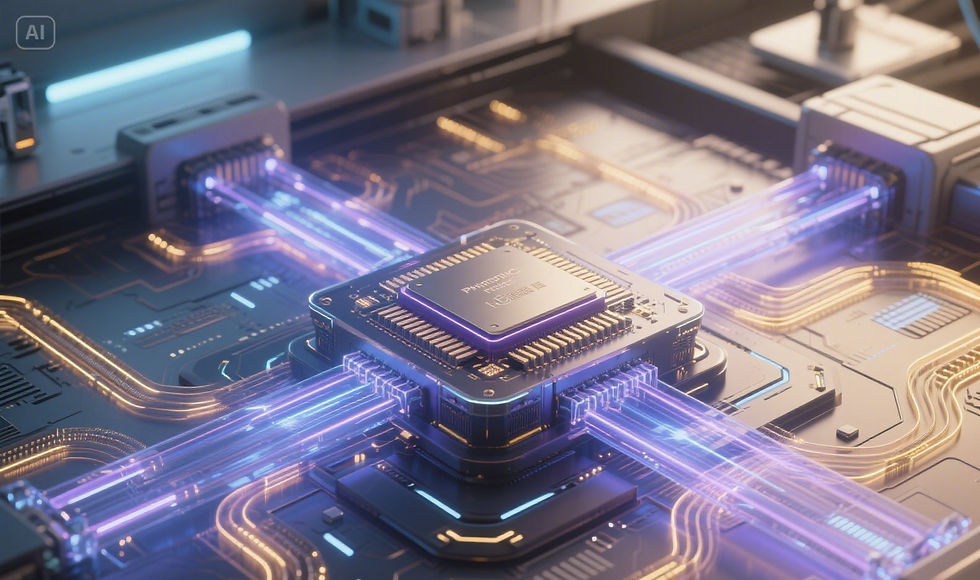
What makes photonic processors stand out is their optical parallelism. Multiple wavelengths can travel simultaneously through the same optical channel, allowing the system to process multiple instructions concurrently with high bandwidth and minimal latency. This makes them ideal for real-time data-intensive tasks such as generative AI, large-scale language model training, and high-performance data centers.
Companies like Lightmatter are leading the field, developing chips that integrate optical circuits directly onto CMOS platforms. Research teams at MIT and the UCSB Photonics Lab are also advancing hybrid solutions, combining photonic logic with electronic control to enhance compatibility with current ecosystems.
Despite the excitement, photonic chips still face critical challenges: high manufacturing costs, miniaturization hurdles, and complex optical signal routing at chip scale. Nonetheless, they remain one of the most tangible bets in the realm of future processors, especially for enterprise and research environments that demand scalable, energy-efficient computing.
2.2 Neuromorphic Processors: Computing Like a Brain
While photonic processors reinvent the physical medium of computing, neuromorphic processors reimagine its logic. Inspired directly by the human brain, these architectures mimic biological structures such as neurons and synapses, prioritizing massive parallelism, adaptive plasticity, and extreme energy efficiency.
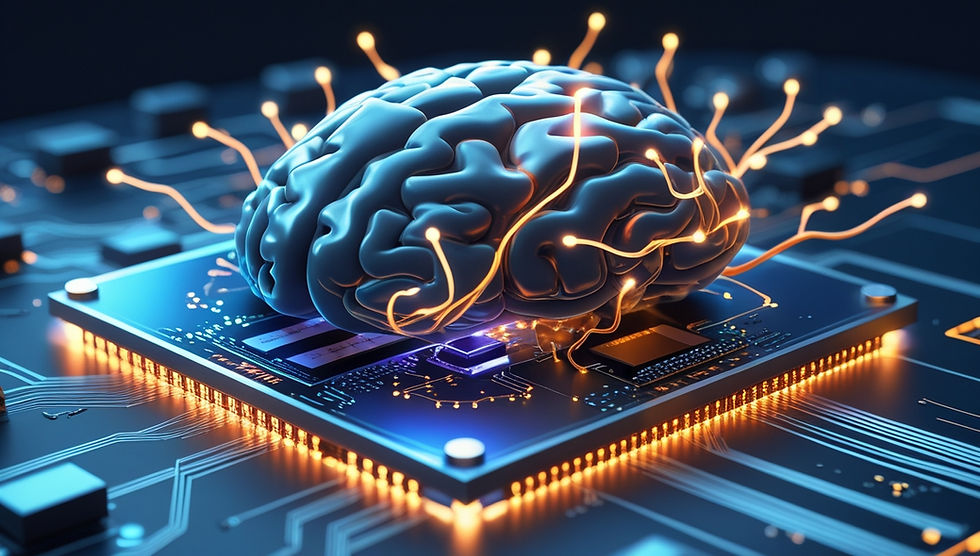
At the core of neuromorphic computing is the encoding of information through sparse electrical pulses—similar to neural “spikes”—and continuous reconfiguration of synaptic connections based on experience. This allows real-time learning directly on the hardware (in-chip learning), enabling applications where latency and power consumption must be minimal, such as in cognitive robotics, embedded systems, and intelligent edge sensors.
Among the current highlights is Intel’s Loihi 2, a chip featuring over one million programmable synapses already capable of pattern recognition and optimization tasks in dynamic environments. IBM's TrueNorth implements one million “artificial neurons” with power consumption under 100 mW. Meanwhile, Australian company BrainChip stands out with its Akida processor, tailored for embedded AI and autonomous vehicles.
Still, this architecture of emerging processor technologies faces challenges to broader adoption. Programming standards are lacking, frameworks remain immature, and compatibility with conventional systems is limited. Yet, their potential is clear—particularly as the demand for distributed, resilient, and energy-efficient AI solutions continues to grow.
2.3 Quantum Processors: The Power of Superposition
Quantum computing doesn’t just redefine the concept of speed — it redefines the very notion of what is computationally possible. Instead of using traditional binary bits (0 or 1), quantum processors operate with qubits, which can exist in multiple states simultaneously through properties like superposition and quantum entanglement. This unlocks the ability to solve problems that are literally impossible for classical computers—such as simulating complex molecules or optimizing high-dimensional systems with billions of variables.
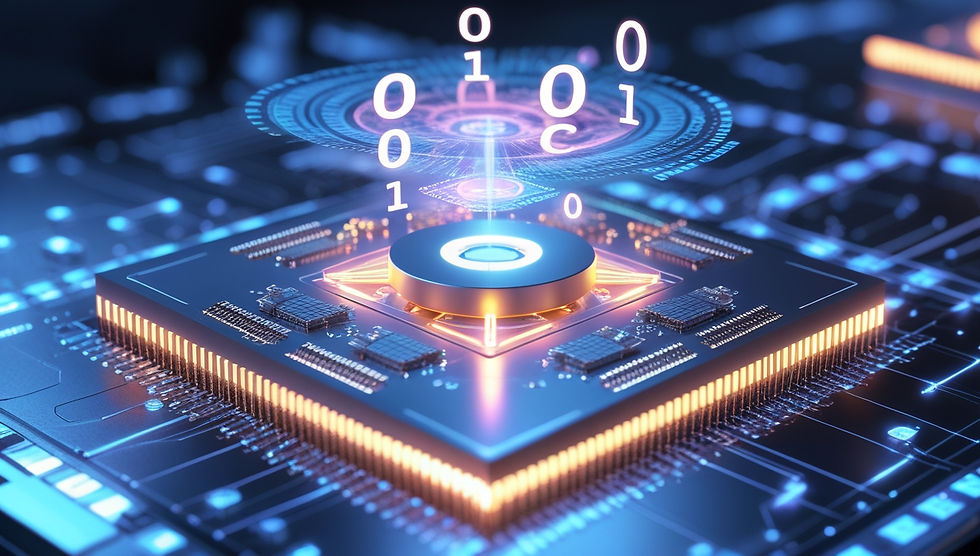
However, this power comes with significant challenges. Qubits are extremely unstable, prone to quantum noise and decoherence, requiring constant error correction and cryogenic environments often colder than -270°C. This makes engineering these next-generation chips a highly specialized field, still far from broad commercial application.
Nonetheless, breakthroughs are advancing rapidly. In 2019, Google’s Sycamore achieved quantum supremacy by solving a problem in 200 seconds that would take a classical supercomputer 10,000 years. IBM Quantum, through its Qiskit-based open platform, leads in cloud-accessible qubits and aims to surpass 1,000 qubits by 2025. Meanwhile, D-Wave and Rigetti Computing are exploring alternative models using quantum annealing and superconducting transmons, targeting applications in optimization and quantum machine learning.
Although still in the early stages of maturity, quantum processors already occupy a strategic position within the ecosystem of emerging processor technologies. They are among the most promising candidates for pushing the boundaries of advanced computing, especially in fields such as drug discovery, post-quantum cryptography, climate modeling, and quantum AI.
2.4 Bioprocessors: Computing with Life
While quantum computing expands the limits of physics, bioprocessors—also known as biological processors—seek inspiration from life itself to reinvent computing at the molecular level. These technologies use biological molecules such as DNA, proteins, or enzymes to process, store, and transmit data. The goal? To build processor architectures that are not only compact and efficient but also self-configuring, biodegradable, and even self-replicating.
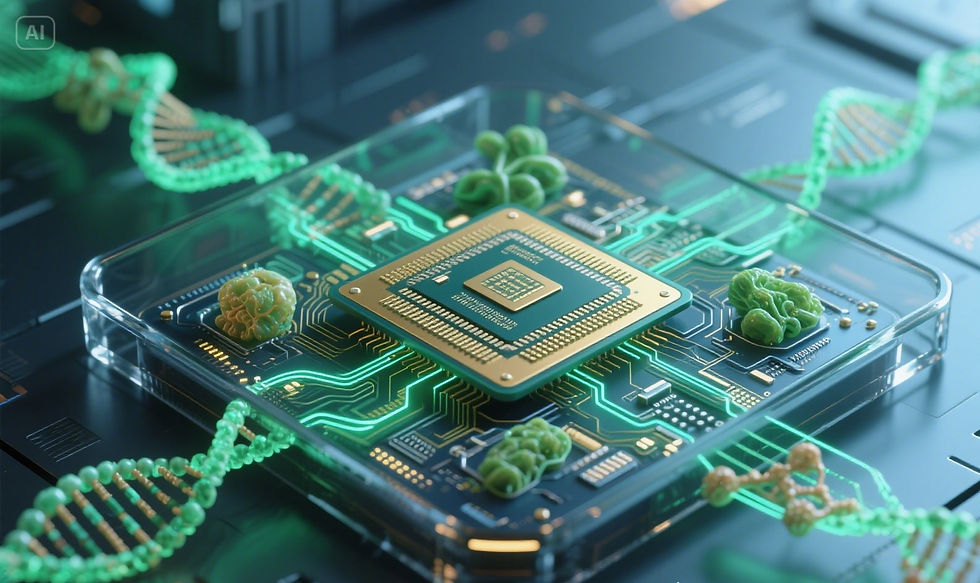
Unlike a traditional transistor, a single strand of DNA can store data with ultra-high density—up to 215 petabytes per gram—far exceeding modern hard drives. Furthermore, molecular recognition and programmable biochemical reactions enable computational logic similar to digital circuits, potentially operating inside living cells.
The most immediate applications include personalized medicine, smart implants, and environmental biocomputing, where biological sensors can respond in real time to internal or external stimuli. At the Harvard Wyss Institute, researchers have created prototypes of DNA logic circuits capable of detecting and neutralizing cancer cells. Meanwhile, institutions like ETH Zurich and Caltech are developing DNA-based chips for biological data analysis and high-precision wearable sensors.
Despite their revolutionary potential, bioprocessors still face core challenges—such as low processing speed, instability in open environments, and the absence of standardized development platforms. For now, they remain a high-value promise in the field of emerging processor technologies, with long-term focus and highly specialized applications.
2.5 Graphene and 2D Material Processors: The Era of Ultraflexible Chips
If silicon is thick, rigid, and constrained by its crystalline structure, graphene and other two-dimensional (2D) materials propose the opposite: ultrathin, flexible chips with superior electronic properties. Graphene, a single layer of carbon atoms arranged in a hexagonal lattice, offers electrical conductivity up to 100 times higher than copper and exceptional electron mobility—features that position it as a strong contender among emerging processor technologies.
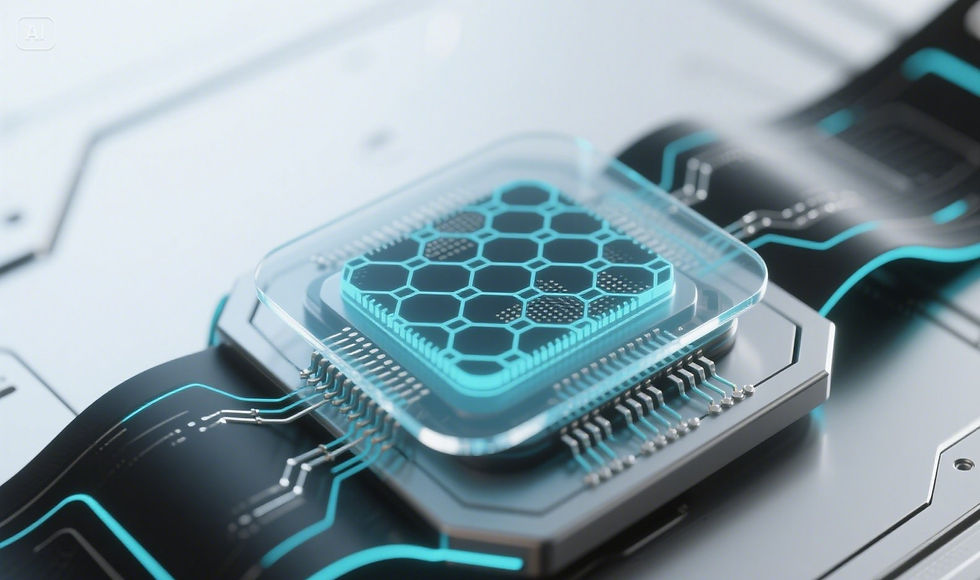
Alongside graphene, materials such as transition metal dichalcogenides (TMDs) and carbon nanotubes expand the design space. These compounds offer unique advantages: tunable semiconductivity, optical transparency, and mechanical elasticity. As a result, next-generation processors based on 2D materials are especially promising for wearables, transparent electronics, bioelectronic sensors, and flexible devices that must be thin, lightweight, and resilient.
The industry is already investing heavily in this transition. IBM has developed graphene transistors operating above 100 GHz. The Samsung Advanced Institute of Technology has secured key patents for integration in foldable displays and biometric sensors. While more conservative, TSMC is partnering with academic institutions like National Taiwan University and ETH Zurich to explore hybrid processor architectures incorporating graphene.
However, graphene-based processors still face significant technical barriers. Large-scale production of uniform layers and reliable integration with industrial substrates remain major challenges. Graphene’s tendency to oxidize in ambient conditions and its low intrinsic bandgap also require advanced engineering solutions.
Despite these limitations, graphene and 2D materials represent one of the most consistent fronts in the transition toward the future of computing. Their role is not to fully replace silicon, but to complement it in hybrid architectures, enabling new categories of devices where flexibility, transparency, and electrical conductivity are essential.
3. Technology Comparison
Below is a comparative overview of the main emerging processor technologies, assessing speed, energy efficiency, current maturity level, and key applications. This table contextualizes how each innovation addresses specific challenges in advanced computing and contributes to the future of computing.
Technology | Speed | Energy Efficiency | Maturity | Key Applications |
Photonic Processors | 🔹 Extremely High | 🔹 High | 🟡 Medium | Generative AI, data centers, optical communication |
Neuromorphic Processors | 🟡 Medium | 🔹 Very High | 🟡 Medium | Cognitive robotics, smart sensors, edge AI |
Quantum Processors | 🔹 Exponential | 🔹 High | 🔴 Low | Cryptography, molecular simulation, advanced research |
Bioprocessors | 🟡 Variable | 🔹 Extremely High | 🔴 Very Low | Personalized medicine, bioengineering, smart implants |
Graphene & 2D Material Processors | 🔹 Very High | 🔹 High | 🟡 Medium | Wearables, transparent/flexible electronics |
5. Market and Challenges
5.1 Market Landscape and Trends
The global semiconductor sector is undergoing a historic transformation. In 2023, the market surpassed $600 billion, and projections by McKinsey & Company and Gartner suggest it will reach $1 trillion by 2030. This growth isn’t solely driven by traditional chip demand but is fueled by the race toward future processors that will enable breakthroughs in AI, advanced computing, biotechnology, and post-quantum cryptography.
With billions in public and private investments, emerging processor technologies have become a strategic focus of global innovation. Initiatives like the CHIPS Act in the U.S., the European Chips Act, and Asia's investments in quantum and neuromorphic computing are shaping the next generation of chips.
Still, the shift will be gradual and hybrid. Silicon will remain relevant due to its maturity and established manufacturing ecosystem, while new processor architectures will address specific performance demands. The future of computing will be defined by the coexistence of multiple paradigms.
5.2 Critical Challenges
Despite growing interest and investment, broad adoption of the technologies discussed in this article faces substantial technical and structural barriers:
• Integration with Current Manufacturing: Many new processors require deep changes in fabrication tools, design software, and test infrastructure. Compatibility with industry standards—built over decades around CMOS—remains limited, especially for quantum chips, bioprocessors, and DNA-based chips.
• Cost and Scalability: Development of photonic, neuromorphic, and graphene processors still demands massive R&D budgets with long-term returns. Scaling up production is a real challenge, particularly for 2D materials and bioinspired structures that lack standardized industrial processes.
• Environmental and Ethical Concerns: The pursuit of more sustainable processor technologies doesn’t eliminate risks. Disposal of graphene devices, chemical reagents in nanofabrication, and manipulation of biological materials in bioprocessors raise concerns about environmental impact, biosafety, and computational ethics—issues that will require clear regulation in the years ahead.
Conclusion
Silicon remains the foundation of the global digital infrastructure, but the signs of its physical and economic limitations are becoming increasingly evident. As we approach the boundaries of miniaturization and thermal efficiency, the need for complementary paths — not necessarily immediate replacements — becomes more urgent.
The technologies explored in this article — photonic processors, neuromorphic chips, quantum computing architectures, bioprocessors, and 2D material-based processors — represent promising lines of research and engineering, each with the potential to meet specific demands of advanced computing. However, all of them still face technical, manufacturing, and regulatory challenges that limit their large-scale adoption in the short term.
The most realistic outlook is a hybrid future for next-generation processors and AI chips, where traditional and emerging technologies will coexist — tailored to the computational task, operating environment, and energy constraints. Progress will depend not only on advances in material science, but also on integration with production ecosystems, new software architectures, and innovation policies that account for both environmental impact and ethical concerns.
The future of computing will not be shaped by a single breakthrough, but by continuous technical evolution, guided by strategic decisions that balance performance, security, sustainability, and economic viability. In this landscape, understanding the possible pathways is just as crucial as recognizing the limits of the technologies we rely on today.
Enjoyed this article? Share it on social media and continue to follow us to stay tuned on the latest in AI, breakthroughs and emerging technologies.
Thanks for your time!😉
Comentarios